
4.0 Active Control Surface Implementation for Studies
on LCO
This section was included to provide a basis for designing active control surfaces to
be used in the current wing-stabilator model of AWT. As a first step in the implementation
process of active control surfaces for LCO, AWT will review active control surfaces used to
suppress flutter. Despite the fact that flutter and LCO are caused by different reasons,
AWT feels that the study of active control surfaces used to suppress flutter is a good place
to start because the goal for both orientations is to prohibit wing tip oscillation. The
implementation schemes of active control surfaces from NASA TR R-450 [7] are analyzed
in this section. The concepts of the implementation scheme will then be viewed in a form
applicable to the current wing/stabilator model.
4.1 Flutter Suppression by Use of Active Control Surfaces
At NASA Langley Research Center in Hampton, VA, a team of engineers sought to
suppress flutter by means of using active controls. Flutter is the self-excited oscillation in
which energy is absorbed by the lifting surface from the airstream [2]. El Nissim's
aerodynamic energy criterion [8] states that sign of the work per cycle done by the system
on the airstream, when the lifting surface undergoes an oscillatory motion, indicates the
state of stability of the system. Nissim stated that a necessary and sufficient condition for
the prevention of flutter is for all oscillatory motions of an elastic system in an airstream to
have positive work done by the system on the surrounding airstream. A brief discussion
concerning key points illustrated by equations given by Nissim, is provided below in order to
grasp his concept of flutter suppression centered on aerodynamic energy considerations.
Consider the equations of motion for a system with n degrees of freedom [8]
[4.1.1]
where, at flutter, the generalized force {F} = 0 and is the circular frequency of oscillation;
[M] is the mass matrix; [Ar] and [AI] are the real and imaginary unsteady aerodynamic-
force matrices, respectively; [K] is the structural stiffness matrix; is the fluid density; S
and b are a reference area and length, respectively; and {q} is the generalized displacement
vector.
Nissim shows that the work per cycle W done by the system on the airstream can be
written as [8]
[4.1.2]
where [8]
[4.1.3]
A positive value for W indicates a transfer of energy from the system to the airstream and
hence, stability. The matrix [U] is Hermitian and therefore possesses real eigenvalues. By
use of these eigenvalues, it is shown in reference 2 that the energy input per cycle into the
airstream can be reduced to a principal quadratic form as [8]
[4.1.4]
where are the eigenvalues of the matrix [U] and denotes generalized coordinates
associated with the aerodynamic energy. A notable characteristic of the energy method is
that the criterion for flutter stability is determined by the characteristics of the
aerodynamic-force matrices alone. Therefore, if a particular system has undesirable flutter
characteristics such as too low of a flutter speed, the flutter characteristics can be
improved if a mechanism can be found which changes the [U] matrix in a appropriate
manner. One such mechanism is the addition of active control surfaces to the basic
system. The motions of these surfaces generate aerodynamic forces that modify the
aerodynamic terms in the [U] matrix for the basic system. For flutter suppression, the
control surface deflections are related by a control law to the plunging and pitching motion
of the main surface. Nissim points out that a suitable configuration is one utilizing both
leading-edge and trailing-edge controls since the two working together would provide
independent control lift and pitching moment.
The wing configuration used in the analytical study of flutter suppression can be seen
in figure 6. Three spanwise locations of aerodynamic control surfaces were considered in
the investigation, designated as II, III, and I in the figure. Each configuration consisted of
both leading-edge and trailing-edge control surfaces activated by sensors located on the
wing at the 30 percent and 70 percent midspan chord of each spanwise location. The
results showed improvement in flutter speed of 21 percent for configuration I, 28 percent in
configuration II, and 11 percent for configuration III. The combined use of configurations II
and I working concurrently produced an improvement in flutter speed in excess of 41
percent.
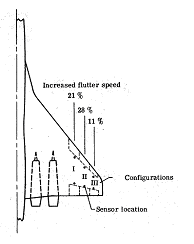
Figure 6. Calculated Effectiveness of Aerodynamic Energy Concept Flutter-Suppression
Systems [7]
The analytical study indicated that active control surfaces were very effective in
increasing flutter speeds; therefore, a research program was undertaken to construct a
model that would incorporate the idea of control surfaces so experimental results could be
compared to the analytical results. The following design was used as a start in AWT's ideas
of implementing control surfaces with an actuating system and power supply.
The design objective was to have a relatively simple and inexpensive model that would
be of adequate size to incorporate control surfaces with an oscillatory drive mechanism.
Figures 7 and 8 show schematic illustrations of the wing model.

Figure 7. Typical Details of Model Construction [7]

Figure 8. A Sketch of the Delta Wing Model with all Dimensions in Meters [7]
For details on the construction of the wing, see NASA TR R-450 [7]. Initially, the
control surfaces were mechanized with an electro-mechanical system. The original
mechanization consisted of a quick-response high-torque electric dc motor, mounted
externally to the model, which was connected to and drove the control surface through a
drive shaft. However, a problem occurred with the wind-up of the long drive shaft. After
researching considerably it was decided to develop a hydraulic servo system so that the
actuator could be located internally, next to the control surface. Prior to installing the new
control system, a static hinge moment test was conducted in the wind tunnel. Measuring
the order of magnitude of the hinge moment was valuable to finalizing the actuator design.
The team had to design and fabricate special actuators to match the desired torque, small
size, and lightweight requirements that the model demanded. A photograph of the actuator
and its components installed in the wing can be seen in the following figure 9 below.

Figure 9. 9. A Mockup of the Actuator System within the Wing [7]
The actuator consisted of a closed compartment separated into two chambers by a self-
sealing vane attached to a shaft and supported by two miniature precision ball bearings.
Shaft rotation is obtained by applying a differential hydraulic pressure between the two
chambers. The actuator has a mass less than 60 g and is capable of providing 4.52 N-m
torque output over the frequency range from 0 to 25 Hz with a 6.9 MPa supply pressure.
The actuator angular displacement capability was approximately . To formulate an
adequate mathematical model to be used in the flutter analysis, the nine structural mode
shapes, generalized masses, and natural frequencies were measured. The mode shapes
were obtained by using a non-contact deflection-measuring device which is described in
detail in A Non-contacting Displacement Measuring Technique and Its Application to
Current Vibration Testing [7].
Using control law theory, an analytical study of the wing model was performed to
determine the best locations for the control surfaces and motion sensors. The procedure,
results, testing, and modifications of the experiment can be seen in NASA TR R-450 [7].
With the analytical study and the experiment on flutter in mind, AWT constructed
similar active controls on a program called Shade. Details of the design are offered in the
next section.
4.2 Constructing Ailerons and Flaps
Figure 10 is a schematic of a possible design for implementing active controls. AWT
plans to add ailerons and flaps to the existing F-111 wing model in WRW 319. The control
surfaces will assist Active Wing Technologies in researching and testing for LCO data. The
following schematic includes leading- and trailing-edge active controls. The trailing-edge
flaps will be constructed inboard and the ailerons will be constructed outboard with trim
tabs fixed on the trailing edge. Spoilers are optional and are conventionally constructed
above the flaps. AWT decided to go with a leading-edge slat as opposed to an trailing edge
flap after realizing the maximum lift coefficient could be increased significantly by this
convention.

Figure 10. A Design of Active Controls by AWT
The tip of the wing model will not include control surfaces. Hydraulic actuators are possibly
the means to displace the active controls. AWT was unable to decide on a power supply for
the active controls but future groups can make a decision when a suitable power supply is
found for the wing sweep. Small hydraulic systems were used as power supplies in the
original experiment conducted by Randall Bolding in his pursuit to find a method of
suppressing flutter. This is a possible system for displacing the control surfaces because a
smaller hydraulic system may not pose as many issues as the hydraulic system used for the
wing sweep. The wing sweep hydraulic system was much larger, outdated, and extremely
loud. Modern hydraulic systems will not have such issues, especially for a much smaller
power requirement. The hydraulic lines can be fed within the wing and will not interfere with
wind tunnel testing. AWT plans to construct the control surfaces by cutting into the wing
model and adding the control surfaces. An alternative method for a power supply is to use
a servo to displace the wing. However, the flaps and ailerons will need a rod in order to be
connected to the power supply. The control surfaces will pivot about the rod and a gear
system could be also of use in order to connect the flaps to the ailerons. The servos can
be simultaneously connected to the wing sweep power supply if necessary. Below is a
schematic of the side view of the wing model.

Figure 11. Control Surface Design
The design of the control surfaces was constructed from existing models used in the air
force such as the F/A-18A. Figure 11 shows the range of rotation of the control surfaces
about the wing. In order to find the power requirement for the actuators or servo system
to be located in the wing model for the control surfaces, the moment will have to be
calculated about the control surfaces. The moment can be calculated through wind tunnel
testing. This is a consideration for future groups continuing the research on LCO
phenomenon.
4.3 Calculating the Hinge Moment
The following section is provided as a supplemental study of flight dynamics. This
section is a starting point or a place to begin in calculating hinge data for determining power
requirements and control surface specifics. The force calculations discussed in this section
is of the elevator and not the flaps itself. Also, the following study pertains exclusively to
the static conditions and the dynamic considerations are left for future groups. The
discussion is taken from Dr. David G. Hull's Introduction to Airplane Flight Mechanics [9].
The air flowing over an elevator creates a pressure distribution on its surface that
triggers a moment about the elevator hinge line called the elevator hinge moment. In order
to maintain the elevator at a specific angle, the pilot must provide a control moment
opposite in sign to the hinge moment by a force on the control column. This can be done
by the use of a hydraulic system if the pilot is not able to move the elevator himself in the
case of a large aircraft. Otherwise, the pilot is directly connected to the elevator so that
he will be able to feel the full affect of the moment acting on the elevator and thus
counteract the moment by force.
To illustrate the analysis of the stick force and hinge moment, consider the reversible
control system in figure 12 system.

Figure 12. The Sign Convention for Control
A positive hinge moment is in the clockwise direction. The stick force can be expressed as
FS=GH [4.3.1]
where G is the gearing and H is the hinge moment. The gearing includes the moment arm
which translates the stick force to a moment. The elevator hinge moment is then changed
to a coefficient by dividing by the dynamic pressure , the elevator area SE, and a mean
elevator chord expressed as follows
[4.3.2]
so that the stick force becomes
[4.3.3]
Next, the hinge moment is assumed to vary linearly with and so that
[4.3.4]
where =0 because tail surfaces are symmetric. Finally, with the tail efficiency factor, the
angle of attack of the horizontal tail, the downwash angle, and equation 4.3.3, the stick force
leads to the following
[4.3.5]
To isolate the dynamic pressure effect, the following expressions are used
[4.3.6]
where . Figure 13 illustrates the stick force vs. the flight speed for low sub-
sonic speeds.

Figure 13. The Stick Force vs. the Flight Speed

|